 |
Information box |
The main purpose of this site is to extend the
intraoperative monitoring to include the neurophysiologic
parameters with intraoperative navigation guided with Skyra 3
tesla MRI and other radiologic facilities to merge the
morphologic and histochemical data in concordance with the
functional data.
CNS Clinic
Located in Jordan Amman near Al-Shmaisani hospital, where all
ambulatory activity is going on.
Contact: Tel: +96265677695, +96265677694.
Skyra running
A magnetom Skyra 3 tesla MRI with all clinical applications
started to run in our hospital in 28-October-2013.
Shmaisani hospital
The hospital where the project is located and running diagnostic
and surgical activity. |
|
 |
|
 |
Evoked potentials are small electrical events arising from
neural tissue occurring in response to abrupt sensory
stimulation. In
current clinical
application this usually involves stimulation of the visual,
auditory, or somatosensory system.
Evoked
potentials were first described in 1875 by Richard Caton who
recorded spontaneous electrical potentials from the cortex of
animals and noted that the spontaneous (electroencephalographic)
activity changed in response to visual stimulation. He also
applied electrical stimuli to peripheral nerves and recorded
"evoked" electrical potentials from appropriate cortical
regions. Among the early discoveries about the human
electroencephalogram (EEG) after Berger's 1929 report was that
photic stimulation gives rise to small potentials in the
occipital region (the driving response) and that potentials
could be elicited by auditory stimulation during sleep
(K-complexes). Subsequent work has shown that there are many
types of responses to stimulation that are timelocked (i.e.,
have a fixed temporal relationship to the stimulus,
however, most
responses are too small to be distinguished from the ongoing
EEG.
An early
approach to resolving the inherent signal-to-noise problem was
reported by George Dawson in 1947. Responses to repetitive
stimuli were displayed on an oscilloscope and superimposed on
photographic film. Time-locked activity produced an overexposure
in one area of the film as compared with random activity, which
lightly exposed all of the film. The technique permitted the
identification of low-amplitude potentials. time-locked to the
stimuli. This superimposition is known as the overtrace method.
Subsequently, the averaging of multiple responses by computer
techniques facilitated the recording of evoked potentials: the
larger random events of the ongoing activity are suppressed by
the averaging process, and smaller evoked events that have a
fixed temporal relationship to a stimulus are preserved.
Availability of commercial systems has facilitated the recording
of sensory evoked potentials in a wide variety of clinical
situations.
 |
General
Clinical Applications |
 |
Evoked potentials are principally useful in neurological
practice for the evaluation of multiple sclerosis, which
in its early stages often has symptoms and/or signs of
one clinical lesion but one or more subclinical lesions
that may be demonstrable by evoked potential techniques.
Many
degenerative disorders of the nervous system are
associated with evoked potential abnormalities. Visual
evoked potentials are often useful in ophthalmologic
assessment, and brain stem auditory evoked responses
provide an objective test of hearing in addition to
their value in the detection of intrinsic or extrinsic
lesions of the brain stem. Brain stem auditory and
somatosensory evoked potentials are also useful in the
assessment of coma and brain death. Some longlatency
evoked potentials are reported to be helpful in the
assessment of dementia and psychogenic disorders.
Somatosensory evoked potentials have the capacity to
evaluate both the peripheral and central nervous systems
from the distal peripheral nerves to the sensory cortex.
Responses
derived from all modalities have been used in monitoring of
operative procedures in which neural tissue is in jeopardy. In
such monitoring, a stimulus is applied distally and responsive
neural tissue is evaluated at a location proximal to the
surgical procedure. The serial responses are evaluated
continuously in reference to baseline values. Physiologic
alteration resulting in evoked potential (EP) changes may warn
of impending irreversible damage that may be averted by
appropriate action.
 |
Evoked
Potential Instrumentation and Techniques |
 |
Analog neural electrical activity is amplified,
filtered, and converted to digital values for
computation and storage. A trigger pulse is supplied by
the stimulator or the computer triggers the stimulator
to establish the time-locked relationship. Each epoch is
stored and added to previously obtained digital values,
and the sum is normalized by the number of stimuli
presented. The average is then displayed on a screen,
stored magnetically, and displayed on paper. The
averaging process results in a marked improvement of the
signal-to-noise ratio, in which the noise reduction
factor can be estimated by calculating
1/√N,
where
N is the number of stimuli delivered to evoke the
average. The number of individual responses required to
form a noise-free average may vary from less than 10 to
over 4000 depending on the relative amplitudes of the
signal and noise. When the signal is large and the noise
is small, few responses are required. Unfortunately, the
opposite is more common, and more than 1000 stimuli may
be needed.
 |
Visual Evoked
Potentials |
 |
In
the current practice of evoked potentials, stimulation
of the visual system may be by flash or pattern (see
below). Flash stimulation of the visual system affords a
diffuse retinal stimulus, activating both the central
and peripheral visual fields. Shifting checkerboard or
grating patterns provide mainly macular stimulation, and
the response is dependent on the integrity of the
central visual field.
Stimulation
to elicit a visual evoked potential requires light with abrupt
onset, such as a stroboscopic light or an array of
lightemitting diodes (LEDs). Flash evoked responses (Fig-1)
consist of a number of positive and negative peaks that vary
greatly between individuals and are affected by subtle changes
in the level of arousal and parameters of stimulation. This
variability has limited their clinical utility. However, they
are helpful in assessing patients whose visual acuity is too
poor to generate evoked potentials to pattern reversal and in
testing comatose patients and others unable to fixate on the
pattern stimulus. Changes in the latencies and morphology of the
responses have been used to follow the course of hydrocephalus
both before and after shunting, with improvement in the flash
evoked potentials. when shunting is successful and regression of
the expected maturational change in the response if shunt
failure occurs. The absence of a flash response is indicative of
poor or absent visual function, and failure to record such
responses in children usually indicates a poor prognosis for
visual development.
Fig-1: Normal
visual evoked potentials (VEP) in response to
unpatterned flash stimulation. Responses recorded
from left occipital (LO), midline occipital (MO),
right occipital (RO), and vertex (V) electrodes.
Occipital electrodes are separated horizontally by 5
cm. 200 stimuli were used to form each each of the
replicated averages. The filter passband was 1 to
100 Hz. The convention for designation of peaks and
valleys is that of Roman numerals. These scalp
recorded potentials are of cortical origin and
demonstrate a feature of near-field responses with
marked changes in polarity and latency as a function
of electrode location. Flash responses, although
robust in amplitude, are unique to each individual,
making identification of abnormality difficult
to impossible. Further, the scalp responses
are dominated by peripheral retinal field
responses, making flash responses insensitive to
common ventral maculofoveal defects. |
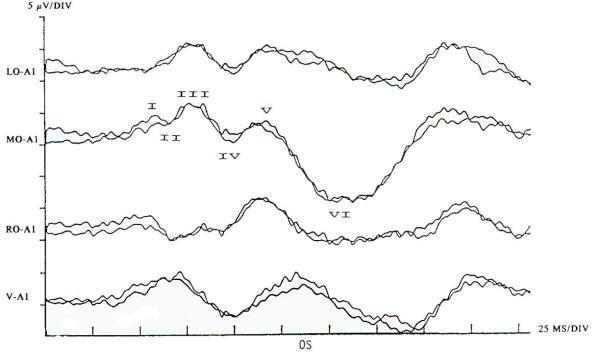 |
Pattern
reversal techniques use several types of stimulators, all of
which provide a checkerboard image. Stimulation occurs when the
black (or colored) squares become white and vice versa. This is
accomplished mechanically by pattern shift or electrically by
pattern reversal. The patient is instructed to fixate on the
shifting pattern. Recording electrodes are placed at scalp
locations over the visual cortex. Prechiasmal disturbances can
be detected by abnormalities of the response from a midline
occipital electrode, with each eye being stimulated separately
(Fig-2). Retrochiasmal lesions require multiple simultaneous
recording channels with electrodes placed over lateral occipital
and posterior temporal regions. The practical utility of evoked
potentials in the assessment of retrochiasmal lesions is open to
debate and requires a high level of patient cooperation (Fig-3).
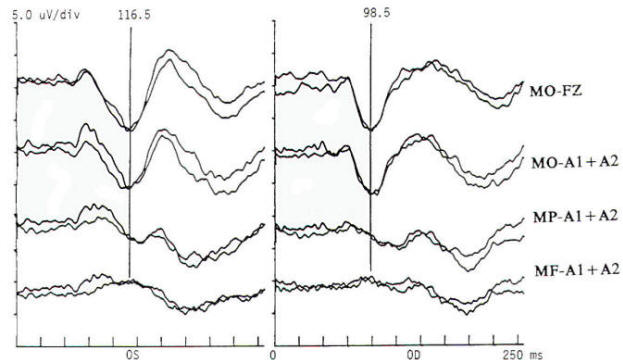 |
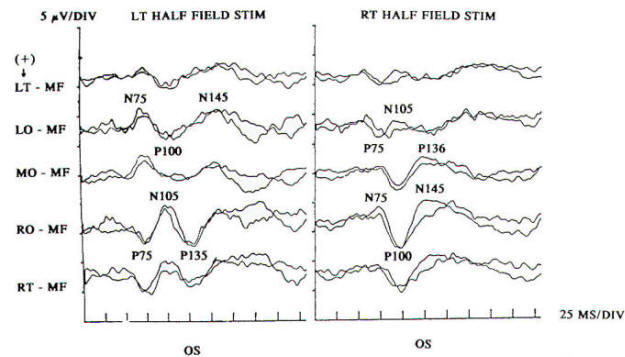 |
Fig-2: A pattern visual evoked potential (PVEP)
study with
markers indicating the P100 component latency in
response to left (OS) and right (OD) eye stimulation.
The OS P100
was identified at 116.5 ms and the right
at 98.5 ms. Thirty-minute arc checks were used reversing
at 3.9/s. Filtering produced a passband of 1 to 100 Hz.
Responses of this case with a history of transient but
totally resolved acuity loss are abnormal. The absolute
latency OS is abnormal as compared to a control
population (upper limit 108 ms for the patient's head
size) and the interocular asymmetry is excessive at 18
ms. Such a conduction delay is suggestive of a
demyelinating process and is compatible with the
clinical diagnosis of multiple sclerosis. |
Fig-3: Normal responses to partial field stimulation
with an appropriate voltage field distribution revealed
by five temporal-occipital electrodes separated
horizontally by 5 cm. There is paradoxical
lateralization of the P100 to the scalp contralateral to
the occipital lobe generating the response. Scalp
ipsilateral to generation reveals a negative potential
(N 105). Electrophysiologists refer to this phenomenon
as the ipsilateral response because left hemifield
stimulation activates the right visual cortex but the
response paradoxically projects to the left scalp, which
is ipsilateral to the stimulated field. Retrochiasmal
lesions will occasionally produce such asymmetric
responses, which can be confirmed by marked attenuation
or absence of the response to appropriate partial field
stimulation. Clinical application of such techniques is
limited because of reduced amplitude of the response
with partial field stimulation and difficulty with
requisite patient cooperation. |
Response
latency and amplitude are sensitive to alteration of technical
parameters and are affected by size and intensity of the
checkerboard pattern, rate of stimulation, filter settings and
head size. The major events of clinical interest occur
within 200 ms in normal individuals. The analysis time is
generally 250 ms and an artifact-free response may require 100
to 400 stimuli.
Criteria
for abnormality related to prechiasmal lesions are
statistically based, primarily on the latency of the P100
component. Pattern reversal evoked responses have a consistent
morphology. The latency of the principal positive component
occurring at about 100 ms (P100) when subjected to statistical
analysis yields a low standard deviation in the range of 2 to 4
ms. A normal pattern reversal response from right eye
stimulation is shown in Fig-2; the negative components occur
around 75 and 145 ms. and are therefore called N75 and N145.
With
prechiasmal lesions, abnormalities of pattern reversal
responses consist primarily of a unilateral or bilateral
prolongation in latency of the P100 component, or an asymmetry
in latency of the P100 between the eyes exceeding statistical
norms. This is usually defined as the mean plus three standard
deviations. A better statistical
criterion is the tolerance limit (TL), in which the 99 percent
TL is calculated from a sample of the normal population.
Although often present, amplitude changes can be affected by non
neurological factors, including simple errors of refraction.
Evoked potential latency prolongation is often seen in multiple
sclerosis and may be present bilaterally when symptoms are
unilateral or even when the patient is visually asymptomatic.
The predilection of multiple sclerosis for the optic nerve is
well known: autopsy series have suggested that as many as 90
percent of multiple sclerosis (MS) patients may have demyelination of the optic nerves even though there may have
been no visual symptoms during life. Evoked potential (EP)
testing reveals almost the same incidence of abnormality in
end-stage MS. An acute attack of optic neuritis may not be
associated with any discernible abnormality of the eye on
clinical examination, and visual acuity not uncommonly returns
to normal, although an enlargement of the central blind spot,
pallor of the optic disc, or impairment of color perception may
be detectable. Early investigators reported that 100 percent of
MS patients who had had optic neuritis showed prolongation of
response latencies, even if they no longer had disturbances of
visual acuity or fields. Although the actual figure may not be
this high, it probably exceeds 90 percent. Moreover, it is
estimated that less than 5 percent of abnormal responses
subsequently return to the normal range. Approximately 60
percent of patients with MS have response abnormalities despite
no history or findings of optic nerve involvement. Thus, visual
evoked potentials are a useful diagnostic tool for detecting
additional lesions of MS that are not evident clinically and for
confirming that subjective complaints of visual disturbance are
in fact due to organic visual dysfunction.
Compressive
or destructive lesions in or around the optic nerve may prolong
response latencies, but they are more likely to attenuate
amplitude or distort morphology. Prominent changes are caused
by a glioma of the optic nerve. Such lesions are not uncommon
in children, and occur less frequently in adults. They are often
subtle in their clinical presentation and may present as visual
complaints of possibly functional character. Diseases of the
eye or optic nerve that primarily affect visual acuity will also
cause diminution of response amplitudes.
 |
Brain Stem
Auditory Evoked
Potentials |
 |
Potentials
evoked by stimulation of the auditory system of normal persons
develop within 2 ms after the stimulus with a cochlear
microphonic and subsequent compound action potential from the
auditory nerve. The later components are generally larger in
amplitude and more variable in morphology. All the components
occurring within the first 7 to 9 ms are of brain stem origin
and are recorded as far-field events with the exception of wave
I.
Middle-latency auditory evoked potentials (20 to 100 ms) may
arise in the brain stem and thalamus, while the frontal and
temporal lobes, particularly the hippocampus, have been
implicated in the generation of long-latency (>
100 ms) auditory evoked
potentials.
Click
stimulation is supplied to the auditory system through
headphones, generally unilaterally and with white noise masking
of the contralateral ear. The electrical impulse is a square
wave of very brief (100 µs) duration. The oscillations it
creates in the headphone contain a broad frequency spectrum
with the major energy in the 3- to 4-kHz range. The latency of
the responses are directly influenced by the intensity of the
click. The clicks are generated by inward or outward movement of
the headphone diaphragm with respect to
the tympanic membrane, and may have one of two polarities:
rarefaction (R), in which the headphone diaphragm initial
movement is away from the tympanic membrane, and condensation
(C), in which movement is toward the tympanic membrane. An
alternating polarity that is an interleaved mixture of the above
R and C clicks is also employed.
Short-latency auditory evoked potentials are now widely used in
neurology and audiology. Seven components may be recorded within
the first 10 ms and are designated by Roman numerals I through
VII. Only waves I, III, and V are considered mandatory
(obligate) for determination of normalcy. Waves II, IV, VI. and
VII may be absent in normal individuals. Wave I is a nerve
action potential of the acoustic nerve, wave III is of lower
pontine generation, and wave V is thought by the majority of
workers in the field to have lower midbrain generation (Fig-4). These are probably ipsilateral responses of
white-matter tracts, rather than the nuclear responses initially
proposed. Waves II, IV, VI. and VII are more variable in terms
of their morphology; waves IV and V may be fused; and waves I
and III may have more than one peak. With the exception of wave
I. these are far-field responses, generated in the brain stem
but recorded at the scalp; wave I is near field, recorded by the
ear or mastoid electrode and generated by the peripheral nervous
system. A brief 100-µs rectangular pulse delivered to
headphones generates a click stimulus having a broad frequency
range from 300 to 6000 Hz. Clicks are generally delivered at
rates of 10 to 80/s. Faster rates of stimulation prolong both
absolute and interpeak latencies. Recordings are usually made
from a vertex-to-ear or -mastoid derivation. Analysis time is 10
ms, and as many as 4000 stimuli may be required to obtain an
artifactfree response. These responses are influenced by a
number of nonpathologic factors. Lower stimulus intensities and
more rapid rates of click presentation produce longer absolute
latencies. Females have shorter latencies than males. Latencies
lengthen with increasing age and decreasing brain temperature,
and are prolonged in neonates, decreasing to adult values by 2
years of age. Latencies are also influenced by the acoustic
phase of the click stimulus. Normality or abnormality is
determined by the presence or absence of waves I, III or V. and
by the interpeak latencies between them as compared with
statistical norms.
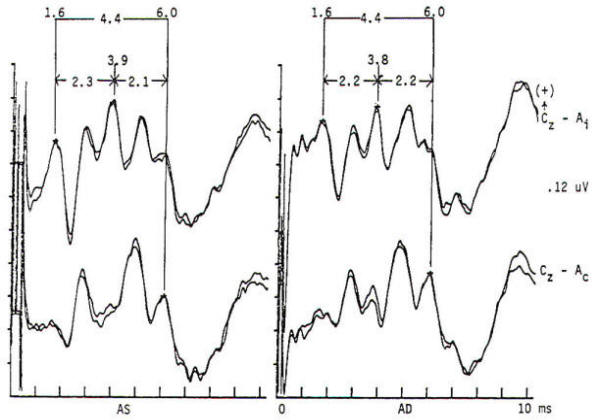 |
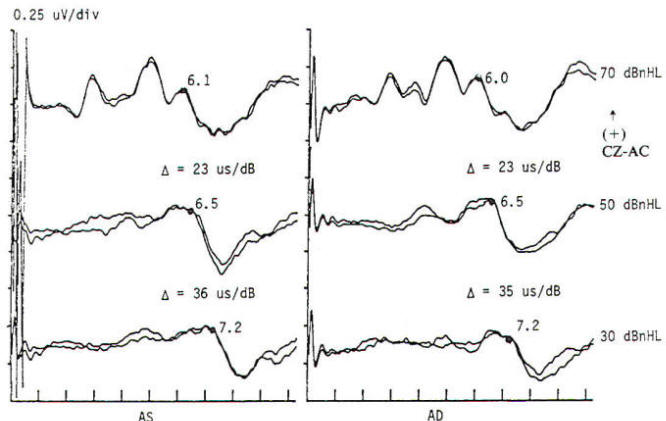 |
Fig-4: Normal brain stem auditory evoked potentials
(BAEP) in a preoperative study of a patient suffering
hemifacial spasm. Brief (100-µs)
electrical pulses produce rarefaction acoustic clicks of
broad-band frequency content (0.2 to 6 kHz). With a
passband of 150 to 3000 Hz, 2000 stimuli form each
average. Obligate waves I (action potential of the
acoustic nerve) occur at 1.6 ms bilaterally, waves III
(of lower pontine generation) occur at 3.9 and 3.8 ms,
and waves V (of lower midbrain generation) occur at 6.0
ms. The I to V interpeak latency is a normal 4.4 ms
bilaterally. The stimulation rate is 51.1/s. Peak
identification is enhanced by distributional criteria
revealed by a two-channel montage. Inspection of the
contralateral derivation (Cz-Ac) reveals the following
differences as compared to the ipsilateral derivation
(Cz-Ai): wave I is absent, wave III is absent or
attenuated, and wave V is enhanced with better IV to V
separation. |
Fig-5: BAEP intensity/latency series obtained by
measuring wave V latency at two or more levels of
stimulus intensity. Wave V (the most robust wave at low
intensity) occurs progressively later as intensity
(dBnHL, decibels normal hearing level) is lowered. Both
conductive and neurosensory deficits may be missed by
single high-intensity stimulation. The latency changes
in µs/dB are
definable for normal and abnormal audiologic conditions.
This "physiologic audiogram" is less sensitive to subtle
deficits than a pure tone audiogram but is free of
subjective bias and can be used in neonates and others
incapable of valid subjective responses. |
Brain stem
response audiometry is a technique of evaluating end-organ
function in patients who cannot participate in behavioral
testing (comatose patients and neonates). This is generally done
by determining the wave V latency as a function of stimulus
intensity (Fig-5). Relatively distinct patterns of response
are produced by patients suffering conductive as opposed to sensorineural dysfunction. Deafness of functional origin is
associated with normal responses, as is deafness produced by
bilateral lesions of the auditory cortex. Generally one cannot
prove organic deafness on the basis of abnormal or absent
responses, although it is often implied. In multiple sclerosis
and some posterior fossa structural lesions, however,
unilaterally absent responses may be encountered in patients
who have no audiologically detectable hearing deficit.
Although
magnetic resonance imaging is now widely used in the diagnosis
of multiple sclerosis, auditory evoked potentials are useful in
patients with negative imaging studies and/or clinical examination. A sizable minority (20 to 40 percent) of MS
patients have brain stem auditory evoked potential (BAEP)
abnormalities, including patients without symptoms or signs of
brain stem dysfunction. Thus, as in visual EPs, the test may
provide physiologic evidence of areas of involvement that are
silent clinically. This may take the form of complete or
partial absence of waveforms, relative attenuation of wave V or
prolonged interpeak latencies
(Fig-6). In a Mayo Clinic study; a significant number
of patients with brain stem evoked potential abnormalities at
the initial presentation, when only a single neurological lesion
was suggested clinically, developed clinically definite MS
within the next 3 years. Response abnormalities of similar
character are also seen in other demyelinating diseases. Chief
among these are the leukodystrophies of childhood, which are
generally hereditary and involve developmental disturbance,
motor dysfunction and central pontine myelinolysis.
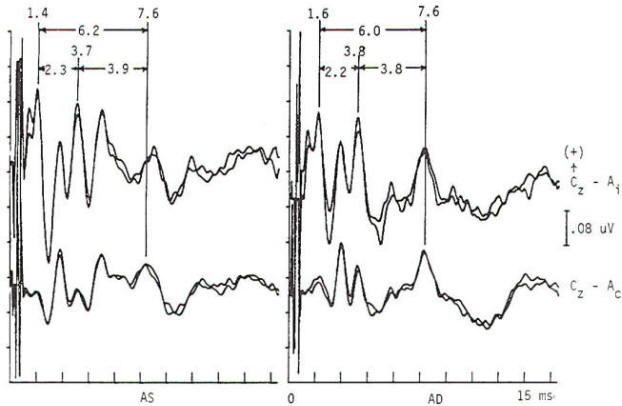 |
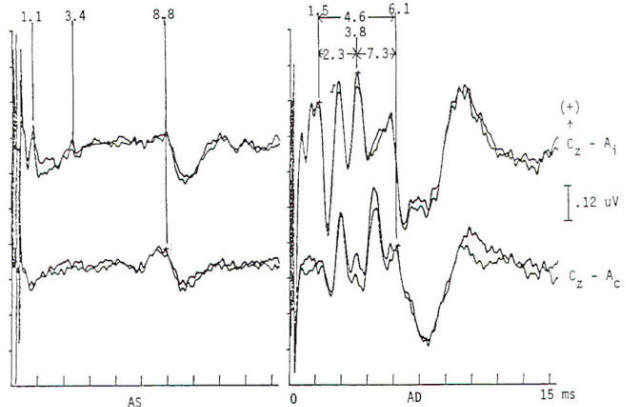 |
Fig-6: BAEP in a preoperative study of a patient with
symptoms of tic douloureux. Responses are normal through
wave III. Wave V is markedly delayed bilaterally and
attenuated (particularly AS). The I to V interpeak
latency is prolonged to 6.2 and 6.0 ms AS and AD,
respectively (upper limit. 4.6 ms). Subsequent brain
magnetic resonance imaging and spinal fluid examination
were consistent with the clinically suspected diagnosis
of multiple sclerosis. |
Fig-7: BAEP responses in a patient presenting with
postural dizziness. AD
responses are entirely normal. AS
responses reveal a sharp component at 1.1 ms, which is
too early to be a wave I and probably represents a
cochlear microphonic. Wave V occurs at 8.8 ms. A small
peak replicates at 3.4 ms and may be a wave I. A pure
tone audiogram was normal. Imaging studies revealed a
mass in the cerebellopontine angle,
that proved at surgery
to be a vestibular schwannoma. BAEPs in CPA tumors vary
greatly in morphology although the responses shown here
are common. |
Intrinsic
or extrinsic tumors of the brain stem may present clinically
with various combinations of cranial nerve, motor tract, and
sensory pathway dysfunction, although a functional diagnosis is
often entertained until the neurological findings become
blatant. EP abnormalities develop early in both intrinsic and
extrinsic brain stem tumors. A wide variety of abnormalities may
be produced by slowly progressive extrinsic tumors, of which
acoustic neuroma is the best example (Fig-7). Such
abnormalities may not only involve prolongation of interpeak
latencies or attenuation of responses on the side of the tumor,
but may also include abnormalities on the opposite side because
of brain stem compression from the neoplasm. Evoked
potential monitoring has been found helpful during surgical
resection of such tumors, in which the facial and acoustic
nerves may be damaged. Changes in evoked potentials have
been associated with intraoperative compromise of acoustic
nerve function, while identification of the facial nerve by
electrophysiologic techniques may help to avoid its sacrifice.
Another
major area of utility is the evaluation of coma. In patients
unconscious as a result of bilateral cerebral cortical
dysfunction, and in other diseases diffusely involving the
cerebral cortex such as Alzheimer's disease, responses are
generally normal. The same is true of psychogenic coma. In coma
due to bilateral damage of brain stem structures, abnormality or
absence of responses indicates a poor prognosis. Brain stem
auditory evoked potentials are resistant to generalized seizures
and anesthesia, and normal responses can be recorded when the
EEG is isoelectric, even when the patient otherwise meets
clinical criteria of brain death. Thus, preservation of BAEP
in a patient with coma, apnea, areflexia and flat EEG may
suggest an overdose if the history is not known when the patient
presents in coma. Alternately, profoundly abnormal or
absent responses support an impression of irreversible brain
insult. In both head-injured patients and those subjected to
ischemia or anoxia, inability to record brain stem auditory
evoked potentials is correlated with a poor outcome: however,
one must be cautious in interpreting such data, as the patient
may have preexisting audiologic disease. Prolonged deep
barbiturate anesthesia is utilized in some centers in the
treatment of severe cerebral trauma. BAEPs have been used to
monitor such patients, as they may be the only evidence of
preserved neural function because vital signs may be suppressed
by the anesthetic agents.
Long-latency evoked potentials may be elicited by auditory,
visual, or somatosensory modalities and may even be "evoked" by
the absence of stereotypical events. As a class they are often
referred to as cognitive potentials or event-related potentials.
They are alleged to be capable of assessing cognitive function
and enable the study of neuropsychiatric disorders. The
best-known and most widely used, the P300 wave, is elicited by
auditory stimulation and has been reported to show prolongation
or attenuation in dementia as compared to the pseudodementia
that often accompanies major depression. Changes in complex
partial as opposed to generalized epilepsy have been described
and P300 abnormalities have been found in patients with
parkinsonism and related disorders who have early dementia.
Preservation of P300 with possible improved prognostic
implications after major traumatic brain injury has been
reported, while P300 latency increases after minor head trauma
have supported the view of an organic origin of some cases of postconcussive syndrome.
 |
Somatosensory Evoked
Potentials |
 |
Stimulation
to produce a somatosensory evoked response
is
usually electrical and is
applied transcutaneously to a peripheral nerve, the amount of
current being the level required to produce a clearly visible
muscle twitch. Although intensity-dependent changes in the
response are present at stimulus levels below motor threshold,
there is little change in amplitude or latency of the response
once the stimulus is above motor twitch threshold.
Electrical stimulation of dermatomes has been reported, which
has the advantage of specific root evaluation. Seyal et al.
reported on the use of segmental spinal EP responses in the
study of lumbosacral root disease. Responses from surface
electrodes over the lower spine were obtained in response to
lower extremity peripheral nerve stimulation. Nerves with
limited root access to the central nervous system (CNS) were
used. In only a minority of patients is useful additional
information gained that is not known from clinical imaging
and electromyographic data. The required EP techniques are
difficult and time consuming. Inadequate data are obtained when
scalp responses to either dermatomal or segmental stimulation
is used. Excessive false-positive and -negative interpretations
were the result of using scalp recorded data. Finally, direct
stimulation of descending motor pathways during surgery and
transcortical magnetic stimulation of the motor cortex may have
some value in intraoperative assessment of spinal cord function
and study of motor pathways.
Electrical
stimulation, which is the most common clinical procedure,
produces ascending volleys up both motor and sensory pathways in
peripheral nerves. This action potential can be easily recorded
by surface electrodes overlying the nerve at various locations.
These peripheral action potentials are subject to a variety of
unwanted variables, such as age, temperature, limb length and interindividual
variation.
Stimulation
of nerves at the wrist (median or ulnar nerve) or ankle
(posterior tibial
nerve) elicits peripheral and CNS responses .Fig-8 and
9). CNS responses may be generated from the spinal cord,
brain stem, and cortex. The variability induced by peripheral
factors mentioned above is minimized if not eliminated by
determining the latency of the response just prior to entry into
the CNS and calculating the interpeak latencies from this
peripheral response to later, more rostrally generated
responses. Such interpeak latencies indicate the central
transmission time and provide the major criteria for normalcy
of a somatosensory study. The peripheral response from Erbs
point (brachial plexus) or lower spinal (cauda equina)
electrodes is analogous to wave I (auditory nerve I
of the BAEP (Fig-8). The remaining components are a mixture of near- and
far-field events.
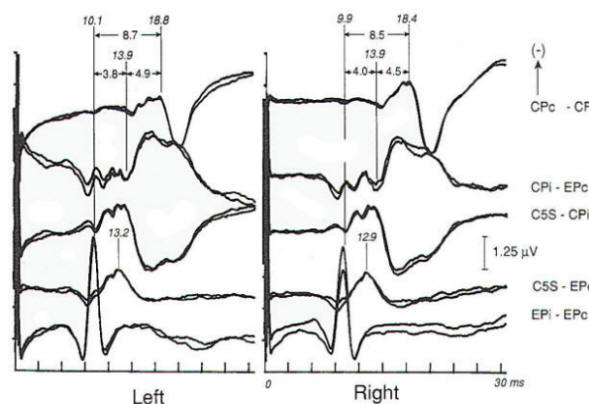 |
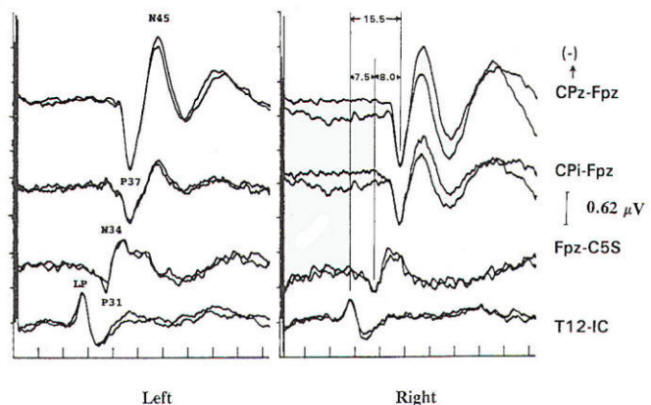 |
Fig-8 Normal median nerve somatosensory evoked
potentials (MN-SSEPs). The bottom channel (EPi-EPc)
demonstrates the Erb's point (EP) response. It is
typically triphasic, with an initial positive, then
negative, then positive complex. Latency is taken from
the negative peak at 10.1 and 9.9 ms following left and
right median nerve stimulation. The second channel from
the bottom (C5S-EPc) records the cervical N13, which is
locally generated from the cervical cord as a horizontal
dipole. It projects posteriorly with negativity and
anteriorly with positivity. Isopotential lines are at
the lateral neck. Latencies are recorded at 13.2 and
12.9 ms. The fourth channel from the bottom is a scalp
to noncephalic reference (CPi-EPc). It records far-field
positive potentials (P9, P11 , P13, and PI4). Of these
only the PI4 is an obligate wave. In this normal patient
it has a latency of 13.9 ms bilaterally. The Pl4 is
thought to arise from postdecussation, medial lemniscal
fibers of the lower brain stem. Pl4 is followed by the
N18 (not labeled), which is a relatively long, slow
potential of subcortical origin. Its site(s) of origin
are disputed, with generation from the medulla to
mesencephalon considered. Here the N18 is seen in
isolation because it is recorded from scalp
contralateral to the cortical N20. The third channel
from the bottom (C5S-CPi) records a confusing
combination of locally generated cervical N13 added to
the far-field P13-P14. Although historically this
derivation has gained wide usage, it can lead to both
false-positive and false-negative interpretations
because N13 and P14 have separate neural generation and
either of these obligate waves may be absent or
temporally dispersed by pathology. In awake patients
with excessive myogenic artifact this channel may have
less artifact than the second and fourth channels. The
top channels (CPc-CPi) demonstrate the cortical N20s at
18.8 and 18.4 ms, respectively. These cortically
generated. near-field potentials are seen in isolation
because the underlying N18 complex, present at both CPc
and CPi, is removed by in-phase cancellation (common
mode rejection). The EP-N20 interpeak latency (IPL) is
often used as the single univariate criterion of
abnormality. Subintervals of EP-N13 or P14 and N13-N20
are used to localize regions of abnormality. (i.
ipsilateral: c. contralateral). |
Fig-9 Normal posterior tibial nerve somatosensory evoked
potentials (PTN-SSEPs). Channels are numbered from top
to bottom. The fourth channel contains the lumbar
potential (LP) recorded with maximal amplitude by a
surface electrode overlying the Tl2 dorsal process
(T12S) and referenced to the iliac crest (IC).
This is a
stationary potential analogous to the median nerve N13
response. Electrodes located several spinous processes
more caudal and cephalad show the component at the same
latency but with lower amplitude. The third channel is a
scalp-cervical noncephalic derivation (FPz-C5S), which
because FPz is out of the field of the cortical
response, records far-field events in isolation.
Components labeled P31 and N34 are far-field
subcortically generated potentials. The P31 is thought
to be analogous to the median nerve Pl4 and the N34
analogous to the N34. These events are not recorded by
the neck electrode. Recording the C5S to hand or foot
indicates the C5S location to be inactive to tibial
stimulation. The second and first channels are
scalp-scalp derivations demonstrating only nearfield
components. The P31 and N34 are not revealed because
they are equipotential at CPZ, CPi, and FPz and are
therefore not amplified because of in-phase
cancellation. The P37 arises from primary sensory cortex
and the N45 has probable generation in association
cortex. Somatosensory topographic organization varies
considerably in normal individuals. The P37 often
projects paradoxically to the contralateral cortex
(similar to the visual P100) and may be absent at the
midline (a severe tilt of the normal vertically oriented
dipole may place the isopotential line through the
vertex). In this patient the P37 amplitude is larger at
the midline (CPZ) than over the lateral (ipsilateral to
stimulation) scalp (CPi). |
The
conduction velocity from the stimulated wrist (median nerve)
to the brachial plexus (or
lower extremity analogue) can and should be calculated, although
because of its long length it is relatively insensitive to
change caused by the acquired peripheral neuropathies, which
primarily involve distal regions. Conduction changes caused by
the various congenital and acquired neuropathies are readily
detected and often present as an unexpected finding in
clinically asymptomatic individuals.
 |
Generators of
Short-Latency Somatosensory Evoked
Potentials |
 |
Several
concepts are helpful to understand the neurophysiologic
assumption underlying many of the assignments of generator
origin. Although
near- and far-field components are present in the visual
and auditory systems, it is in the somatosensory system that
some understanding of the concepts is particularly important for
clinical interpretation. Near-field components are those
waveforms arising from neural tissue close to the recording
electrode. Such responses are relatively large, are slow in both
frequency and latency. and show marked variation in morphology
as recorded from different locations over the scalp (topographic
specificity). In contrast, a far-field response is considered
to be generated by subcortical structures, distant from the
recording electrodes. In biological systems, far-field responses
are relatively small (submicrovolt) and are faster in both
frequency and latency, in addition to showing relative
topographic nonspecificity across the scalp with nearly
identical morphologies at widely separate locations. It is often
this latter feature that is the most striking, such that even
quite large components may be isopotential and cause
cancellation in scalpscalp derivations.
The following is a
discussion of obligate
components seen in response to stimulation of
major compound nerves of upper and lower extremities. Their
neural origin is discussed.
Responses to Upper Extremity Stimulation.
Erb's point: The Erbs point response
is a peripheral nerve action potential recorded from the brachial plexus.
It
has a triphasic positive-negative-positive waveform
characteristic of any peripheral nerve action potential. With a
conduction velocity of
50 to 60 m/s the nerve volley travels from wrist (median.
radial, or ulnar nerve) to the recording site at Erb's point.
Spinal N13-P13 (near-field) These
potentials are best investigated
by the use of electrodes on the posterior cervical region in
conjunction with electrodes on the anterior neck or in the
esophagus. This is a local potential with a horizontal dipole, negative posteriorly and positive anteriorly,
which is thought by Desmedt to be due to a segmental
generator, probably in the dorsal horns. This
potential can be observed in certain lesions that interrupt the
dorsal column pathways and obliterate the other positive
far-field potentials recorded at the scalp. Lueders et al.
point out that N12 (probably equivalent to N13 of Desmedt)
"most probably corresponds to the postsynaptic dorsal cord
potential."
P13 and P14:
These two components probably represent lemniscal activity.
Desmedt and coworkers believe their onset relates to activity in
the caudal most lemniscal fibers, and the peak of P14 corresponds
to arrival of the volley at the thalamus. These far-field
potentials are seen in a cephalic-noncephalic reference and are
not recorded below the level of the foramen magnum. Some authors
have attributed them to activity in dorsal column nuclei but
because of the closed electrical fields generated in nuclear
masses, this seems less likely to many investigators.
The
interpretation of these potentials as arising in lemniscal
pathways would fit with an estimated conduction velocity of
these pathways of 58 m/s and including a synaptic delay of 0.4
ms in the dorsal column nuclei. Further, P14 is preserved in
patients with thalamic lesions that abolish subsequent
scalp-scalp components following stimulation of the
contralateral limb. There has always been some controversy about
the origin of these waves, however, Lueders and coworkers
suggest that P13 originates in ipsilateral dorsal column
pathways at the level of the cervicomedullary junction. They
thought that this far-field potential was due to the abrupt
change in current flow produced when the afferent volley enters
the skull.
N18: This
is a negative potential that begins as early as 16 ms after the
stimulus, and persists for 6 ms or more. It is a far-field
potential (anomalous in that most far-field potentials are
positive and of higher frequency), recorded widely throughout
the scalp. It is preserved after thalamic lesions and is thought
to arise in the brain stem. A similar potential was
recorded by a depth electrode implanted in the fourth ventricle
by Hashimoto. It is
noted the persistence of this potential in patients who suffered a rightsided pontine stroke, even though near-field, scalp-scalp
potentials from that side were obliterated. This potential
probably arises from collateral or reverberating circuits in the
rhombencephalon.
N20: This
potential is thought to arise from the posterior bank of the
central fissure contralateral to the stimulated limb. This is a
near-field potential, typically recorded in scalp-scalp
derivations. For some time it was thought that N20 was simply
the negative end of a dipole, the positive end of which was the
P22 or other variously named positive waves noted below. Desmedt and others have argued that this notion is
untenable.
P22: This
wave is also generated in cortical structures, but is more
widespread, seen throughout the frontal cortex bilaterally. It
peaks 1 or 2 ms later than the more localized parietal
negativity (N20) noted above, and may arise from a direct
connection between the thalamus and the prerolandic cortex.
Later
Potentials: Later potentials are well known, and include P27,
N30, and P45.
They are clearly
state dependent. The generators of many later potentials are
usually attributed to activity in cortical association areas.
Responses
to Lower Extremity Stimulation
Popliteal
Fossa (PF) Potential: The PF potential is a triphasic compound
nerve action potential recorded in response to tibial nerve
stimulation. It is obtained by recording the ascending volley in
the popliteal fossa by bipolar or referential derivations. The
latency is directly dependent on peripheral nerve conduction
velocity and limb length. Because of the difficulty of
recording spinal responses in some patients, the PF potential
is often used to calculate an interpeak latency to later
subcortical and cortical arising potentials. Such interpeak
latencies have smaller variance and therefore greater clinical
utility than the absolute latencies of scalp potentials (P37,
N45 ).
Lumbar Potentials (LP): In derivations using a recording
electrode over the lumbar spine and a distant reference
electrode (the iliac crest, umbilicus, high thoracic), a
biphasic wave is recorded, usually with an absolute latency in
the range of 18 to 25 ms following stimulation of the tibial
nerve at the ankle. The potential is composed primarily of a
stationary component that is maximal in amplitude at the level
of the T12 vertebra. The latency does not change when recorded
from electrodes above and below T12 but the amplitude is
reduced. The component has a dipole configuration with
negativity projected anteriorly. A small traveling wave of
dorsal column origin can be detected and traced when sequential
spinal electrodes at multiple sites are used. This potential is
frequently used as a reference for measurements of central
conduction in the spinal pathways. The EP guidelines of the
American Electroencephalographic Society
refer to this potential as the
lumbar potential (LP) and suggest a derivation from the T12
vertebra referenced to the iliac crest (T12S-IC).
Subcortically Originating Potentials: Desmedt and Cheron have
recorded a positive potential in scalp-noncephalic derivations
that they term the P17, attributed to the peripheral nerve
volley as it enters the sacral plexus at the upper buttock. This
may be analogous to the P9 recorded after median nerve
stimulation. A P21 component noted by Desmedt and Cheron is
attributed to ascending dorsal column activity. They think that
this is the far-field representation of the local N21, the
near-field dorsal column volley. Lueders et al. identify a P27
that they attribute to dorsal column activity as it "enters the
skull at the level of the foramen magnum and is therefore
equivalent to PI3. Other authors recognize a positive
far-field potential occurring at about 30 ms absolute latency
after tibial nerve stimulation. Lemniscal activity seems to
be one of several likely candidates for generation of this
potential.
In
scalp-noncephalic derivations such as FPz-C5S, one may avoid the
superimposed cortical response by selecting an electrode site
out of the field of the cortical response (see below) and record
far-field components in isolation. A P31, analogous to the
median nerve somatosensory evoked potential (MN-SSEP) P13, and
N34, analogous to the MN-SSEP N18, are the most notable.
Apparently because of their subthalamic origin they are far
less influenced by inhalational anesthetic agents and therefore
are often used during intraoperative monitoring.
Cortically
Originating Potentials: The major cortical response is a positive
wave termed P38
by Desmedt and Bourguet. P37
by Chiappa and Ropper. This wave undoubtedly represents the cortical generator
in the leg-receiving area at the edge of the interhemispheric
fissure. It is often of highest amplitude a centimeter or two
lateral to the midline, ipsilateral to the side of stimulation.
This paradoxical lateralization is proposed to be due to a
dipole projection. The hemisphere contralateral to the
stimulated limb generates the response: however, the positive
component of the dipole projects to the opposite scalp area.
Subsequent
potentials (N49. P57. N75. etc.) are similar to later potentials
discussed under median nerve stimulation, are of uncertain
generator source.
Usually the later the latency of the peak or valley. the greater
the variability. This is true not only of morphology and latency
but also of symmetry, as easily demonstrated by poor
reproducibility. Later components are affected by alcohol,
sedatives. tranquilizers, and analgesics as well as level of
arousal and psychological factors. It is this latter fact that
makes the later components of interest to the physiological
psychiatrists and psychologists but limits their value in
neurological diagnosis. Abnormality in these later components
is usually produced by cortical disease, and other diagnostic
procedures such as electroencephalography and imaging studies
are usually of greater diagnostic value.
Following
median nerve stimulation, the Erbs point to N20 latency is
calculated as the overall central conduction time from brachial
plexus to primary sensory cortex. If this value is prolonged as
compared with normative data, further analysis is required to
determine if the prolongation is due to the more caudal Erbs
point to P14 segment or the more rostral P14 to N20 (Fig-10).
In severe lesions
components may be entirely missing or grossly attenuated and
altered in morphology.
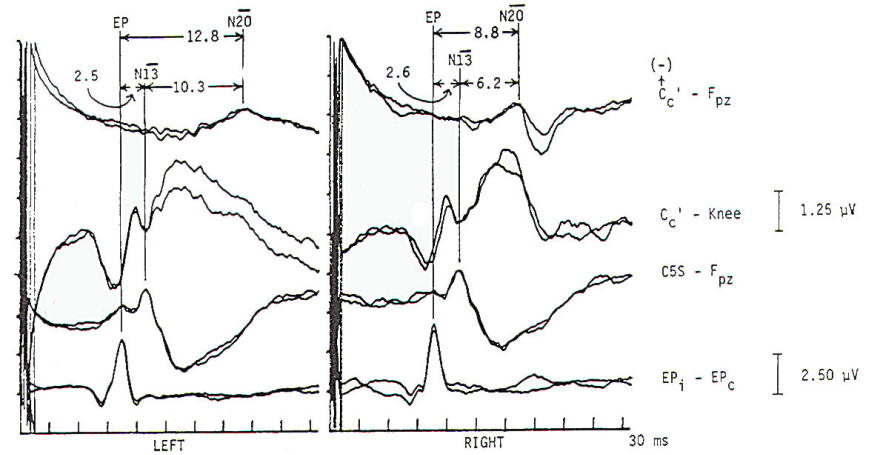 |
Fig-10 Median nerve somatosensory responses demonstrate
symmetric and normal Erbs point (EP) responses and N/Pl3
near- and far-field responses. and grossly asymmetric
N20 responses. EP-N20 IPLs are asymmetric by 4.0 ms
(upper limit is 1.5 ms). Delay occurs between the
cervical/lower brain stem N/Pl3 and the cortically
generated N20. Findings are etiologically nonspecific
but anatomically implicate upper brain stem-thalamic
pathways subserving the medial lemniscal tracts.
Demyelination (conduction delay) rather than conduction
block (voltage attenuation) may be inferred. |
Numerous
reports indicate that the somatosensory evoked potentials are
similar to visual and auditory responses in the sense that they
are sensitive to subclinical lesions. Evidence to support this
contention may be found in the many cases of acute trauma with
clear neurological signs of deficit that resolve with time but
leave subclinical physiologic residuals detectable by the abovedescribed techniques. Somatosensory testing is
reported to be essentially equal to visual EPs in the detection
of occult lesions in MS and to have a higher incidence of
abnormality than auditory brain stem responses in patients
suspect for demyelinating disorders.
The
clinical utility of SSEPs is similar to that of auditory and
visual EPs with some important additions. In MS, somatosensory
responses are equally sensitive for the identification of occult
lesions as compared to pattern reversal visual EPs and more
sensitive than auditory brain stem responses. Neoplasia of
the spinal cord and brain stem usually produces major
disturbances. Although rarely necessary in the evaluation of
brain death, somatosensory evoked potentials have a certain
advantage over BAEPs because the stimulus has direct access to
the nervous system rather than being transduced by the cochlea,
which may be defective for many reasons.
Obviously,
this form of stimulation is at least theoretically capable of
evaluating both the peripheral and central nervous systems from
toe to cortex (excluding the spinothalamic pathways). Peripheral
neuropathies may be detected. Useful information may be obtained
in patients suffering traumatic brachial plexopathies in which
the degree of root involvement is unknown. Amyotrophic
lateral sclerosis was initially thought to have no effect on
sensory EPs but it is now recognized that lower extremity SSEPs
may be abnormal in 40 percent of such patients. The use of SSEPs in
evaluating basic mechanisms of spinal cord and nerve
transmission, as well as response to therapy, has been well
demonstrated.
|
 |
Starting from July-2007 all the surgical activities of
Prof. Munir Elias will be guided under the electrophysiologic control of
ISIS- IOM
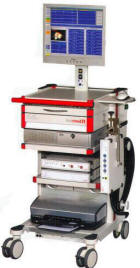
ISIS-IOM Inomed Highline

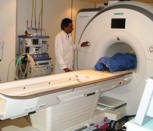
Starting from 28-November-2013 Skyra with all clinical applications in
the run. |
|